Principles of Biochemistry/Chromosome and its structure
![]() |
This page was imported and needs to be de-wikified. Books should use wikilinks rather sparsely, and only to reference technical or esoteric terms that are critical to understanding the content. Most if not all wikilinks should simply be removed. Please remove {{dewikify}} after the page is dewikified. |
In a series of experiments beginning in the mid-1880s, Theodor Boveri gave the definitive demonstration that chromosomes are the vectors of heredity. His two principles were the continuity of chromosomes and the individuality of chromosome. It is the second of these principles that was so original.Wilhelm Roux suggested that each chromosome carries a different genetic load. Boveri was able to test and confirm this hypothesis. Aided by the rediscovery at the start of the 1900s of Gregor Mendel's earlier work, Boveri was able to point out the connection between the rules of inheritance and the behaviour of the chromosomes. Boveri influenced two generations of American cytologists: Edmund Beecher Wilson, Walter Sutton and Theophilus Painter were all influenced by Boveri (Wilson and Painter actually worked with him).
In his famous textbook The Cell in Development and Heredity, Wilson linked together the independent work of Boveri and Sutton (both around 1902) by naming the chromosome theory of inheritance the "Sutton-Boveri Theory" (the names are sometimes reversed). Ernst Mayr remarks that the theory was hotly contested by some famous geneticists: William Bateson, Wilhelm Johannsen, Richard Goldschmidt and T.H. Morgan, all of a rather dogmatic turn-of-mind. Eventually, complete proof came from chromosome maps in Morgan's own lab.[1]


Chromosome
[edit | edit source]A chromosome is an organized structure of DNA and protein that is found in nucleus of the cell. It is a single piece of coiled DNA containing many genes, regulatory elements and other nucleotide sequences. Chromosomes also contain DNA-bound proteins, which serve to package the DNA and control its functions. Chromosomes vary widely between different organisms. The DNA molecule may be circular or linear, and can be composed of 10,000 to 1,000,000,000 nucleotides in a long chain. Typically, eukaryotic cells (cells with nuclei) have large linear chromosomes and prokaryotic cells (cells without defined nuclei) have smaller circular chromosomes, although there are many exceptions to this rule. Also, cells may contain more than one type of chromosome; for example, mitochondria in most eukaryotes and chloroplasts in plants have their own small chromosomes.[2]
In eukaryotes, nuclear chromosomes are packaged by proteins into a condensed structure called chromatin. This allows the very long DNA molecules to fit into the cell nucleus. The structure of chromosomes and chromatin varies through the cell cycle. Chromosomes are the essential unit for cellular division and must be replicated, divided, and passed successfully to their daughter cells so as to ensure the genetic diversity and survival of their progeny. Chromosomes may exist as either duplicated or unduplicated. Unduplicated chromosomes are single linear strands, whereas duplicated chromosomes (copied during synthesis phase) contain two copies joined by a centromere. Compaction of the duplicated chromosomes during mitosis and meiosis results in the classic four-arm structure (pictured to the right). Chromosomal recombination plays a vital role in genetic diversity. If these structures are manipulated incorrectly, through processes known as chromosomal instability and translocation, the cell may undergo mitotic catastrophe and die, or it may unexpectedly evade apoptosis leading to the progression of cancer. In practice "chromosome" is a rather loosely defined term. In prokaryotes and viruses, the term genophore is more appropriate when no chromatin is present. However, a large body of work uses the term chromosome regardless of chromatin content. In prokaryotes, DNA is usually arranged as a circle, which is tightly coiled in on itself, sometimes accompanied by one or more smaller, circular DNA molecules called plasmids. These small circular genomes are also found in mitochondria and chloroplasts, reflecting their bacterial origins. The simplest genophores are found in viruses: these DNA or RNA molecules are short linear or circular genophores that often lack structural proteins. The word chromosome comes from the Greek χρῶμα (chroma, colour) and σῶμα (soma, body) due to their property of being very strongly stained by particular dyes.[3]
Chromatin
[edit | edit source]Chromatin is the combination of DNA, histone, and other proteins that make up chromosomes. It is found inside the nuclear envelope of eukaryotic cells. It is divided between heterochromatin (condensed) and euchromatin (extended) forms. The functions of chromatin are to package DNA into a smaller volume to fit in the cell, to strengthen the DNA to allow mitosis and meiosis, and to control gene expression and DNA replication. Changes in chromatin structure are affected by chemical modifications of histone proteins, such as methylation and acetylation, and by other DNA-binding proteins.
Packaging of DNA in chromatin
[edit | edit source]Chromatin undergoes various forms of change in its structure. Histone proteins, the foundation blocks of chromatin, are modified by various post-translational modification to alter DNA packing. Acetylation results in the loosening of chromatin and lends itself to replication and transcription. When certain residues are methylated they hold DNA together strongly and restrict access to various enzymes. A recent study showed that there is a bivalent structure present in the chromatin: methylated lysine residues at location 4 and 27 on histone 3. It is thought that this may be involved in development; there is more methylation of lysine 27 in embryonic cells than in differentiated cells, whereas lysine 4 methylation positively regulates transcription by recruiting nucleosome remodeling enzymes and histone acetylases[4].[5]
Polycomb-group proteins play a role in regulating genes through modulation of chromatin structure.[6]
Chromatin and Watson/Crick base pairing
[edit | edit source]Crick and Watson's famous structure of DNA (called B-DNA) is only one of three possible structural forms.
For the C-N bond between a base and its sugar there are two different conformations. The anti-conformation occurs in all A- and B-DNAs as well as in Z-DNA where a Cytosine is present. In case of a Guanine Z-DNA takes the syn-conformation. The periodic change between a purine and pyrimidine along the strand of a Z-DNA accomplishes the alternating syn-anti-conformation characteristic of the zigzag structure of the Z-DNA helix.

Histone:The DNA binding protein
[edit | edit source]Histones were discovered in 1884 by Albrecht Kossel. The word "histone" dates from the late 19th century and is from the German "Histon", of uncertain origin: perhaps from Greek histanai or from histos. Until the early 1990s, histones were dismissed by most as inert packing material for eukaryotic nuclear DNA, based in part on the "ball and stick" models of Mark Ptashne and others who believed transcription was activated by protein-DNA and protein-protein interactions on largely naked DNA templates, as is the case in bacteria. During the 1980s, work by Michael Grunstein demonstrated that eukaryotic histones repress gene transcription, and that the function of transcriptional activators is to overcome this repression. We now know that histones play both positive and negative roles in gene expression, forming the basis of the histone code. The discovery of the H5 histone appears to date back to 1970's, and in classification it has been grouped with H1.[7]
Histones are found in the nuclei of eukaryotic cells, and in certain Archaea, namely Euryarchaea, but not in bacteria. Archaeal histones may well resemble the evolutionary precursors to eukaryotic histones. Histone proteins are among the most highly conserved proteins in eukaryotes, emphasizing their important role in the biology of the nucleus.:939 In contrast mature sperm cells largely use protamines to package their genomic DNA, most likely because this allows them to achieve an even higher packaging ratio. Core histones are highly conserved proteins, that is, there are very few differences among the amino acid sequences of the histone proteins of different species. Linker histone usually has more than one form within a species and is also less conserved than the core histones. There are some variant forms in some of the major classes. They share amino acid sequence homology and core structural similarity to a specific class of major histones but also have their own feature that is distinct from the major histones. These minor histones usually carry out specific functions of the chromatin metabolism. For example, histone H3-like CenpA is a histone only associated with the centromere region of the chromosome. Histone H2A variant H2A.Z is associated with the promoters of actively transcribed genes and also involved in the prevention of the spread of silent heterochromatin. Another H2A variant H2A.X binds to the DNA with double strand breaks and marks the region undergoing DNA repair. Histone H3.3 is associated with the body of actively transcribed genes.[8][9]
The nucleosome and "beads-on-a-string"
[edit | edit source]The basic repeat element of chromatin is the nucleosome, interconnected by sections of linker DNA, a far shorter arrangement than pure DNA in solution.
In addition to the core histones, there is the linker histone, H1, which contacts the exit/entry of the DNA strand on the nucleosome. The nucleosome core particle, together with histone H1, is known as a chromatosome. Nucleosomes, with about 20 to 60 base pairs of linker DNA, can form, under non-physiological conditions, an approximately 10 nm "beads-on-a-string" fibre.
The nucleosomes bind DNA non-specifically, as required by their function in general DNA packaging. There are, however, large DNA sequence preferences that govern nucleosome positioning. This is due primarily to the varying physical properties of different DNA sequences: For instance, adenosine and thymine are more favorably compressed into the inner minor grooves. This means nucleosomes can bind preferentially at one position approximately every 10 base pairs (the helical repeat of DNA)- where the DNA is rotated to maximise the number of A and T bases that will lie in the inner minor groove.
What is a Nucleosome? Nucleosomes are the basic unit of DNA packaging in eukaryotes, consisting of a segment of DNA wound around a histone protein core. This structure is often compared to thread wrapped around a spool.
Nucleosomes form the fundamental repeating units of eukaryotic chromatin, which is used to pack the large eukaryotic genomes into the nucleus while still ensuring appropriate access to it (in mammalian cells approximately 2 m of linear DNA have to be packed into a nucleus of roughly 10 µm diameter). Nucleosomes are folded through a series of successively higher order structures to eventually form a chromosome; this both compacts DNA and creates an added layer of regulatory control which ensures correct gene expression. Nucleosomes are thought to carry epigenetically inherited information in the form of covalent modifications of their core histones. The nucleosome hypothesis was proposed by Don and Ada Olins in 1974 and Roger Kornberg. The nucleosome core particle consists of approximately 147 base pairs of DNA wrapped in 1.67 left-handed superhelical turns around a histone octamer consisting of 2 copies each of the core histones H2A, H2B, H3, and H4. Core particles are connected by stretches of "linker DNA", which can be up to about 80 bp long. Technically, a nucleosome is defined as the core particle plus one of these linker regions; however the word is often synonymous with the core particle. Linker histones such as H1 and its isoforms are involved in chromatin compaction and sit at the base of the nucleosome near the DNA entry and exit binding to the linker region of the DNA. Non-condensed nucleosomes without the linker histone resemble "beads on a string of DNA" under an electron microscope. In contrast to most eukaryotic cells, mature sperm cells largely use protamines to package their genomic DNA, most likely to achieve an even higher packaging ratio.Histone equivalents and a simplified chromatin structure have also been found in Archea, proving that eukaryotes are not the only organisms that use nucleosomes.
30 nm chromatin fibre
[edit | edit source]
Left: 1 start helix "solenoid" structure.
Right: 2 start loose helix structure.
Note: the histones are omitted in this diagram - only the DNA is shown.
With addition of H1, the "beads-on-a-string" structure in turn coils into a 30 nm diameter helical structure known as the 30 nm fibre or filament. The precise structure of the chromatin fibre in the cell is not known in detail, and there is still some debate over this.
This level of chromatin structure is thought to be the form of euchromatin, which contains actively transcribed genes. EM studies have demonstrated that the 30 nm fibre is highly dynamic such that it unfolds into a 10 nm fiber ("beads-on-a-string") structure when transversed by an RNA polymerase engaged in transcription.

Linker DNA in yellow and nucleosomal DNA in pink.
The existing models commonly accept that the nucleosomes lie perpendicular to the axis of the fibre, with linker histones arranged internally. A stable 30 nm fibre relies on the regular positioning of nucleosomes along DNA. Linker DNA is relatively resistant to bending and rotation. This makes the length of linker DNA critical to the stability of the fibre, requiring nucleosomes to be separated by lengths that permit rotation and folding into the required orientation without excessive stress to the DNA. In this view, different length of the linker DNA should produce different folding topologies of the chromatin fiber. Recent theoretical work, based on electron-microscopy images[10] of reconstituted fibers support this view.[11]
Spatial organization of chromatin in the cell nucleus
[edit | edit source]The layout of the genome within the nucleus is not random - specific regions of the genome have a tendency to be found in certain spaces. Specific regions of the chromatin are enriched at the nuclear membrane, while other regions are bound together by protein complexes. The layout of this is not, however, well characterised apart from the compaction of one of the two X chromosomes in mammalian females into the Barr body. This serves the role of permanently deactivating these genes, which prevents females getting a 'double dose' relative to males. The extent to which the inactive X is actually compacted is a matter of some controversy.

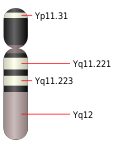
Human chromosomes
[edit | edit source]Chromosomes can be divided into two types—autosomes, and sex chromosomes. Certain genetic traits are linked to your sex, and are passed on through the sex chromosomes. The autosomes contain the rest of the genetic hereditary information. All act in the same way during cell division. Human cells have 23 pairs of large linear nuclear chromosomes, (22 pairs of autosomes and one pair of sex chromosomes) giving a total of 46 per cell. In addition to these, human cells have many hundreds of copies of the mitochondrial genome. Sequencing of the human genome has provided a great deal of information about each of the chromosomes. Below is a table compiling statistics for the chromosomes, based on the Sanger Institute's human genome information in the Vertebrate Genome Annotation (VEGA) database.[12] Number of genes is an estimate as it is in part based on gene predictions. Total chromosome length is an estimate as well, based on the estimated size of unsequenced heterochromatin regions.
An autosome is a chromosome that is not a sex chromosome; that is to say, there is an equal number of copies of the chromosome in males and females.[13] For example, in humans, there are 22 pairs of autosomes. In addition to autosomes, there are sex chromosomes, to be specific: X chromosome and Y chromosome. So, humans have 23 pairs of chromosomes.
Sex chromosomes The X chromosome is one of the two sex-determining chromosomes in many animal species, including mammals (the other is the Y chromosome). It is a part of the XY sex-determination system and X0 sex-determination system. The X chromosome was named for its unique properties by early researchers, which resulted in the naming of its counterpart Y chromosome, for the next letter in the alphabet, after it was discovered later.
The Y-chromosome is one of the two sex-determining chromosomes in most mammals, including humans. In mammals, it contains the gene SRY, which triggers testis development if present. The human Y-chromosome is composed of about 60 million base pairs. DNA in the Y-chromosome is passed from father to son, and Y-DNA analysis may thus be used in genealogy research.

Chromosome | Genes | Total bases | Sequenced bases |
---|---|---|---|
1 | 4,220 | 247,199,719 | 224,999,719 |
2 | 1,491 | 242,751,149 | 237,712,649 |
3 | 1,550 | 199,446,827 | 194,704,827 |
4 | 446 | 191,263,063 | 187,297,063 |
5 | 609 | 180,837,866 | 177,702,766 |
6 | 2,281 | 170,896,993 | 167,273,993 |
7 | 2,135 | 158,821,424 | 154,952,424 |
8 | 1,106 | 146,274,826 | 142,612,826 |
9 | 1,920 | 140,442,298 | 120,312,298 |
10 | 1,793 | 135,374,737 | 131,624,737 |
11 | 379 | 134,452,384 | 131,130,853 |
12 | 1,430 | 132,289,534 | 130,303,534 |
13 | 924 | 114,127,980 | 95,559,980 |
14 | 1,347 | 106,360,585 | 88,290,585 |
15 | 921 | 100,338,915 | 81,341,915 |
16 | 909 | 88,822,254 | 78,884,754 |
17 | 1,672 | 78,654,742 | 77,800,220 |
18 | 519 | 76,117,153 | 74,656,155 |
19 | 1,555 | 63,806,651 | 55,785,651 |
20 | 1,008 | 62,435,965 | 59,505,254 |
21 | 578 | 46,944,323 | 34,171,998 |
22 | 1,092 | 49,528,953 | 34,893,953 |
X (sex chromosome) | 1,846 | 154,913,754 | 151,058,754 |
Y (sex chromosome) | 454 | 57,741,652 | 25,121,652 |
Total | 32,185 | 3,079,843,747 | 2,857,698,560 |
What is nucleoid?
[edit | edit source]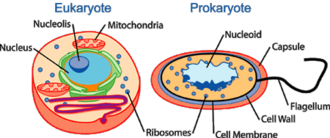
The nucleoid (meaning nucleus-like) is an irregularly-shaped region within the cell of prokaryotes which has nuclear material without a nuclear membrane and where the genetic material is localized. The genome of prokaryotic organisms generally is a circular, double-stranded piece of DNA, of which multiple copies may exist at any time. The length of a genome widely varies, but generally is at least a few million base pairs. Storage of the genome within a nucleoid can be contrasted against that within eukaryotes, where the genome is packed into chromatin and sequestered within a membrane-enclosed organelle called the nucleus. A genophore is the DNA of a prokaryote. This is commonly referred to as a prokaryotic chromosome. The term chromosome is misleading for a genophore because the genophore lacks chromatin. The genophore is compacted through a mechanism known as supercoiling, whereas a chromosome is compacted via chromatin. The genophore is circular in most prokaryotes, and linear in very few. The circular nature of the genophore allows replication to occur without telomeres. Genophores are generally of a much smaller size than Eukaryotic chromosomes. A genophore of a true organism can be as small as 580,073 base pairs (Mycoplasma genitalium). Many eukaryotes (such as plants and animals) carry genophores in organelles such as mitochondria and chloroplasts. These organelles are very similar to true prokaryotes.[14]
Experimental evidence suggests that the nucleoid is largely composed of DNA, about 60%, with a small amount of RNA and protein. The latter two constituents are likely to be mainly messenger RNA and the transcription factor proteins found regulating the bacterial genome. Proteins helping to maintain the supercoiled structure of the nucleic acid are known as nucleoid proteins or nucleoid-associated proteins and are distinct from histones of eukaryotic nuclei. In contrast to histones, the DNA-binding proteins of the nucleoid do not form nucleosomes, in which DNA is wrapped around a protein core. Instead, these proteins often use other mechanisms to promote compaction such as DNA looping.[15]
Circular bacterial chromosome
[edit | edit source]Most bacterial chromosomes contain a circular DNA molecule - there are no free ends to the DNA. Free ends would otherwise create significant challenges to cells with respect to DNA replication and stability. Cells that do contain chromosomes with DNA ends, or telomeres (most eukaryotes), have acquired elaborate mechanisms to overcome these challenges. However, a circular chromosome can provide other challenges for cells. After replication, the two progeny circular chromosomes can sometimes remain interlinked or tangled, and they must be resolved so that each cell inherits one complete copy of the chromosome during cell division.[16]
Structure of Eukaryotic chromosome
[edit | edit source]Some sequences are required for a properly functioning chromosome: Centromere: Used during cell division as the attachment point for the spindle fibers. Telomere: Used to maintain chromosomal integrity by capping off the ends of the linear chromosomes. This region is a microsatellite, but its function is more specific than a simple tandem repeat.
Centromere
[edit | edit source]A centromere is a region of DNA typically found near the middle of a chromosome where two identical sister chromatids come closest in contact. It is involved in cell division as the point of mitotic spindle attachment. The sister chromatids are attached all along their length, but they are closest at the centromere.
The centromeric DNA is normally in a heterochromatin state, which is essential for the recruitment of the cohesin complex that mediates sister chromatid cohesion after DNA replication as well as coordinating sister chromatid separation during anaphase. In this chromatin, the normal histone H3 is replaced with a centromere-specific variant, CENP-A in humans. The presence of CENP-A is believed to be important for the assembly of the kinetochore on the centromere. CENP-C has been shown to localise almost exclusively to these regions of CENP-A associated chromatin. In human cells, the histones are found to be most enriched for H4K20me3 and H3K9me3 which are known heterochromatic modifications. In the yeast Schizosaccharomyces pombe (and probably in other eukaryotes), the formation of centromeric heterochromatin is connected to RNAi. In nematodes such as Caenorhabditis elegans, some plants, and the insect orders Lepidoptera and Hemiptera, chromosomes are "holocentric", indicating that there is not a primary site of microtubule attachments or a primary constriction, and a "diffuse" kinetochore assembles along the entire length of the chromosome.
Centromere positions
[edit | edit source]Each chromosome has two arms, labeled p (the shorter of the two) and q (the longer). The p arm is named for "petit" meaning 'small'; the q arm is named q simply because it follows p in the alphabet("q" refers to the French word "queue" meaning 'tail'.) They can be connected in either metacentric, submetacentric, acrocentric or telocentric manner.
Metacentric
[edit | edit source]A chromosome is metacentric if its two arms are roughly equal in length. In some cases, a metacentric chromosome is formed by balanced Robertsonian translocation: the fusion of two acrocentric chromosomes to form one metacentric chromosome.
Submetacentric
[edit | edit source]If arms' lengths are unequal, the chromosome is said to be submetacentric
Acrocentric
[edit | edit source]If the p (short) arm is so short that is hard to observe, but still present, then the chromosome is acrocentric (The "acro-" in acrocentric refers to the Greek word for "peak".). The human genome includes five acrocentric chromosomes: 13, 14, 15, 21 and 22.
In an acrocentric chromosome the p arm contains genetic material including repeated sequences such as nucleolar organizing regions, and can be translocated without significant harm, as in a balanced Robertsonian translocation. The domestic horse genome includes one metacentric chromosome that is homologous to two acrocentric chromosomes in the conspecific but undomesticated Przewalski's horse.[17] This may reflect either fixation of a balanced Robertsonian translocation in domestic horses or, conversely, fixation of the fission of one metacentric chromosome into two acrocentric chromosomes in Przewalski's horses. A similar situation exists between the human and great ape genomes; in this case, because more species are extant, it is apparent that the evolutionary sequence is a reduction of two acrocentric chromosomes in the great apes to one metacentric chromosome in humans.
Telocentric
[edit | edit source]A telocentric chromosome's centromere is located at the terminal end of the chromosome. Telomeres may extend from both ends of the chromosome. For example, the standard house mouse karyotype has only acrocentric chromosomes.[18] Humans do not possess telocentric chromosomes. Some authors denote extreme acrocentric chromosomes as telocentric- 21, 22, Y.
Subtelocentric
[edit | edit source]If chromosome's centromere is located closer to its end than to its center, it may be described as subtelocentric.
Holocentric
[edit | edit source]With holocentric chromosomes, the entire length of the chromosome acts as the centromere. Examples of this type of centromere can be found scattered throughout the plant and animal kingdoms[19] with the most well known example being in the nematode, Caenorhabditis elegans.
Telomere
[edit | edit source]A telomere is a short and specific region of repetitive DNA at the end of a linear eukaryotic chromosome, which protects the end of the chromosome from deterioration. Its name is derived from the Greek nouns telos "end" and merοs "part". The telomere regions deter the degradation of genes near the ends of chromosomes by allowing for the shortening of chromosome ends, which necessarily occurs during chromosome replication.In 1975–1977, Elizabeth Blackburn, working as a postdoctoral fellow at Yale University with Joseph Gall, discovered the unusual nature of telomeres, with their simple repeated DNA sequences composing chromosome ends. Their work was published in 1978. The telomere shortening mechanism normally limits cells to a fixed number of divisions, and animal studies suggest that this is responsible for aging on the cellular level and sets a limit on lifespans. Telomeres protect a cell's chromosomes from fusing with each other or rearranging — abnormalities that can lead to cancer — and so cells are destroyed when their telomeres are consumed. Most cancers are the result of "immortal" cells that have ways of evading this programmed destruction.
The maintenance of tolomeres depends on a specialized ribonucleoprotein (RNP) which is named tolomerase. Telomeres and tolomerase have different physical behavior but are given their substrate-enzyme relation which forces them to establish a productive interaction. However, the regulatory mechanism that controls their interaction still remains unknown.[20]
Elizabeth Blackburn, Carol Greider, and Jack Szostak were awarded the 2009 Nobel Prize in Physiology or Medicine for the discovery of how chromosomes are protected by telomeres and the enzyme telomerase.
Structure and functions of telomere
[edit | edit source]Telomeres are repetitive DNA sequences located at the termini of linear chromosomes of most eukaryotic organisms, and a few prokaryotes. Telomeres compensate for incomplete semi-conservative DNA replication at chromosomal ends. The protection against homologous recombination (HR) and non-homologous end joining (NHEJ) constitutes the essential “capping” role of telomeres that distinguishes them from DNA double-strand breaks (DSBs).[21]
In most prokaryotes, chromosomes are circular and, thus, do not have ends to suffer premature replication termination. A small fraction of bacteria l chromosomes (such as those in Streptomyces and Borrelia) are linear and possess telomeres, which are very different from those of the eukaryotic chromosomes in structure and functions. The known structures of bacterial telomeres take the form of proteins bound to the ends of linear chromosomes, or hairpin loops of single-stranded DNA at the ends of the linear chromosomes.[22]
In most multicellular eukaryotic organisms, telomerase is active only in germ cells, stem cells and certain white blood cells. There are theories that claim that the steady shortening of telomeres with each replication in somatic (body) cells may have a role in senescence and in the prevention of cancer. This is because the telomeres act as a sort of time-delay "fuse", eventually running out after a certain number of cell divisions and resulting in the eventual loss of vital genetic information from the cell's chromosome with future divisions.
Telomere length varies greatly between species, from approximately 300 to 600 base pairs in yeast[23] to many kilobases in humans, and usually is composed of arrays of guanine-rich, six- to eight-base-pair-long repeats. Eukaryotic telomeres normally terminate with 3′ single-stranded-DNA overhang, which is essential for telomere maintenance and capping. Multiple proteins binding single- and double-stranded telomere DNA have been identified.[24] These function in both telomere maintenance and capping. Telomeres form large loop structures called telomere loops, or T-loops. Here, the single-stranded DNA curls around in a long circle stabilized by telomere-binding proteins.[25] At the very end of the T-loop, the single-stranded telomere DNA is held onto a region of double-stranded DNA by the telomere strand disrupting the double-helical DNA and base pairing to one of the two strands. This triple-stranded structure is called a displacement loop or D-loop.[26]
Telomere shortening in humans can induce replicative senescence, which blocks cell division. This mechanism appears to prevent genomic instability and development of cancer in human aged cells by limiting the number of cell divisions. However, shortened telomeres impair immune function which might also increase cancer susceptibility.[27] Malignant cells that bypass this arrest become immortalized by telomere extension due mostly to the activation of telomerase, the reverse transcriptase enzyme responsible for synthesis of telomeres. However, 5–10% of human cancers activate the Alternative Lengthening of Telomeres (ALT) pathway, which relies on recombination-mediated elongation.
Since shorter telomeres are thought to be a cause of poorer health and aging, this raises the question of why longer telomeres are not selected for to ameliorate these effects. A prominent explanation suggests that inheriting longer telomeres would cause increased cancer rates (e.g. Weinstein and Ciszek, 2002). However, a recent literature review and analysis [27] suggests this is unlikely, because shorter telomeres and telomerase inactivation is more often associated with increased cancer rates, and the mortality from cancer occurs late in life when the force of natural selection is very low. An alternative explanation to the hypothesis that long telomeres are selected against due to their cancer promoting effects is the "thrifty telomere" hypothesis which suggests that the cellular proliferation effects of longer telomeres causes increased energy expenditures.[27] In environments of energetic limitation, shorter telomeres might be an energy sparing mechanism.
Human telomeres and cancer
[edit | edit source]Human somatic cells lacking telomerase gradually lose telomeric sequences as a result of incomplete replication (Counter et al., 1992). As human telomeres grow shorter, eventually cells reach the limit of their replicative capacity and progress into senescence. Senescence involves p53 and pRb pathways and leads to the arrest of cell proliferation. It is thought that senescence plays an important role in suppression of emergence of cancer, although inheriting shorter telomeres probably does not protect against cancer (Eisenberg, 2011).[28] With critically shortened telomeres, further cell proliferation can be achieved by inactivation of p53 and pRb pathways. Cells entering proliferation after inactivation of p53 and pRb pathways undergo crisis. Crisis is characterized by gross chromosomal rearrangements and genome instability, and almost all cells die. Rare cells emerge from crisis immortalized through telomere elongation by either activated telomerase or ALT (Colgina and Reddel, 1999; Reddel and Bryan, 2003). The first description of an ALT cell line demonstrated that the telomeres are highly heterogeneous in length and predicted a mechanism involving recombination (Murnane et al., 1994). Subsequent studies have confirmed a role for recombination in telomere maintenance by ALT (Dunham et al., 2000), however the exact mechanism of this pathway is yet to be determined. ALT cells produce abundant t-circles, possible products of intratelomeric recombination and t-loop resolution (Tomaska et al., 2000; 2009; Cesare and Griffith, 2004; Wang et al., 2004).
Telomerase is a "ribonucleoprotein complex" composed of a protein component and an RNA primer sequence that acts to protect the terminal ends of chromosomes. The actions of telomerase are necessary because, during replication, DNA polymerase can synthesize DNA in only a 5' to 3' direction and can do so only by adding polynucleotides to an RNA primer that has already been placed at various points along the length of the DNA. These RNA strands must later be replaced with DNA. This replacement of the RNA primers is not a problem at origins of replication within the chromosome because DNA polymerase can use a previous stretch of DNA 5' to the RNA template as a template to backfill the sequence where the RNA primer was; at the terminal end of the chromosome, however, DNA polymerase cannot replace the RNA primer because there is no position 5' of the RNA primer where another primer can be placed, nor is there DNA upstream that can be used as a primer so that DNA polymerase can replace the RNA primer. Without telomeres at the end of DNA, this genetic sequence at the end of the chromosome would be deleted and the chromosome would grow shorter and shorter in subsequent replications. The telomere prevents this problem by employing a different mechanism to synthesize DNA at this point, thereby preserving the sequence at the terminal of the chromosome. This prevents chromosomal fraying and prevents the ends of the chromosome from being processed as a double-strand DNA break, which could lead to chromosome-to-chromosome telomere fusions. Telomeres are extended by telomerases, part of a protein subgroup of specialized reverse transcriptase enzymes known as Telomerase reverse transcriptase(TERT) (TElomerase Reverse Transcriptases) that are involved in synthesis of telomeres in humans and many other, but not all, organisms. However, because of DNA replication mechanisms, oxidative stress, and, because TERT expression is very low in many types of human cells, the telomeres of these cells shrink a little bit every time a cell divides, although, in other cellular compartments that require extensive cell division, such as stem cells and certain white blood cells, TERT is expressed at higher levels and telomere shortening is partially or fully prevented.

In addition to its TERT protein component, telomerase also contains a piece of template RNA known as the TERC (TElomerase RNA Component) or TR (Telomerase RNA). In humans, this TERC telomere sequence is a repeating string of TTAGGG, between 3 and 20 kilobases in length. There are an additional 100-300 kilobases of telomere-associated repeats between the telomere and the rest of the chromosome. Telomere sequences vary from species to species, but, in general, one strand is rich in G with fewer Cs. These G-rich sequences can form four-stranded structures (G-quadruplexes), with sets of four bases held in plane and then stacked on top of each other with either a sodium or a potassium ion between the planar quadruplexes.
If telomeres become too short, they have the potential to unfold from their presumed closed structure. It is thought that the cell detects this uncapping as DNA damage and then enters cellular senescence, growth arrest, or apoptosis, depending on the cell's genetic background (p53 status). Uncapped telomeres also result in chromosomal fusions. Since this damage cannot be repaired in normal somatic cells, the cell may even go into apoptosis. Many aging-related diseases are linked to shortened telomeres. Organs deteriorate as more and more of their cells die off or enter cellular senescence.
At the very distal end of the telomere is a 300 bp single-stranded portion, which forms the T-Loop. This loop is analogous to a knot, which stabilizes the telomere, preventing the telomere ends from being recognized as break points by the DNA repair machinery. Should non-homologous end joining occur at the telomeric ends, chromosomal fusion will result. The T-loop is held together by seven known proteins, the most notable ones being TRF1, TRF2, POT1, TIN1, and TIN2, collectively referred to as the shelterin complex.
A study published in the May 3, 2005 issue of the American Heart Association journal Circulation found that weight gain and increased insulin resistance are correlated with greater telomere shortening over time.
Group | Organism | Telomeric repeat (5' to 3' toward the end) |
---|---|---|
Vertebrates | Human, mouse, Xenopus | TTAGGG |
Filamentous fungi | Neurospora crassa | TTAGGG |
Slime moulds | Physarum, Didymium | TTAGGG |
Dictyostelium | AG(1-8) | |
Kinetoplastid protozoa | Trypanosoma, Crithidia | TTAGGG |
Ciliate protozoa | Tetrahymena, Glaucoma | TTGGGG |
Paramecium | TTGGG(T/G) | |
Oxytricha, Stylonychia, Euplotes | TTTTGGGG | |
Apicomplexan protozoa | Plasmodium | TTAGGG(T/C) |
Higher plants | Arabidopsis thaliana | TTTAGGG |
Green algae | Chlamydomonas | TTTTAGGG |
Insects | Bombyx mori | TTAGG |
Roundworms | Ascaris lumbricoides | TTAGGC |
Fission yeasts | Schizosaccharomyces pombe | TTAC(A)(C)G(1-8) |
Budding yeasts | Saccharomyces cerevisiae | TGTGGGTGTGGTG (from RNA template) or G(2-3)(TG)(1-6)T (consensus) |
Saccharomyces castellii | TCTGGGTG | |
Candida glabrata | GGGGTCTGGGTGCTG | |
Candida albicans | GGTGTACGGATGTCTAACTTCTT | |
Candida tropicalis | GGTGTA[C/A]GGATGTCACGATCATT | |
Candida maltosa | GGTGTACGGATGCAGACTCGCTT | |
Candida guillermondii | GGTGTAC | |
Candida pseudotropicalis | GGTGTACGGATTTGATTAGTTATGT | |
Kluyveromyces lactis | GGTGTACGGATTTGATTAGGTATGT |
Mini and micro satellite
[edit | edit source]Satellite DNA consists of very large arrays of tandemly repeating, non-coding DNA. Satellite DNA is the main component of functional centromeres, and form the main structural constituent of heterochromatin. The name "satellite DNA" refers to how repetitions of a short DNA sequence tend to produce a different frequency of the nucleotides adenine, cytosine, guanine and thymine, and thus have a different density from bulk DNA - such that they form a second or 'satellite' band when genomic DNA is separated on a density gradient.
Types of satellite DNA
[edit | edit source]Satellite DNA, together with minisatellite and microsatellite DNA, constitute the tandem repeats.
Some types of satellite DNA in humans are:
Type | Size of repeat unit (bp) | Location |
---|---|---|
α (alphoid DNA) | 171 | All chromosomes |
β | 68 | Centromeres of chromosomes 1, 9, 13, 14, 15, 21, 22 and Y |
Satellite 1 | 25-48 | Centromeres and other regions in heterochromatin of most chromosomes |
Satellite 2 | 5 | Most chromosomes |
Satellite 3 | 5 | Most chromosomes |
Minisatellite
A minisatellite is a section of DNA that consists of a short series of bases 10–60 bp.These occur at more than 1,000 locations in the human genome. Some minisatellites contain a central (or "core") sequence of letters “GGGCAGGANG” (where N can be any base) or more generally a strand bias with purines (adenosine (A) and guanine (G)) on one strand and pyrimidines (cytosine (C) and thymine (T)) on the other. It has been proposed that this sequence per se encourages chromosomes to swap DNA. In alternative models, it is the presence of a neighbouring cis-acting meiotic double-strand break hotspot which is the primary cause of minisatellite repeat copy number variations. Somatic changes are suggested to result from replication difficulties (which might include replication slippage, among other phenomena). When such events occur, mistakes are made, thus causing minisatellites at over 1000 locations in a person's genome to have slightly different numbers of repeats, thereby making each individual unique. The most highly mutable minisatellite locus described so far is CEB1 (D2S90) described by Vergnaud.[29]Minisatellites have been associated with chromosome fragile sites and are proximal to a number of recurrent translocation breakpoints. Some human minisatellites (~1%) have been demonstrated to be hypermutable, with an average mutation rate in the germline higher that 0.5% up to over 20%, making them the most unstable region in the human genome known to date. While other genomes (mouse, rat and pig) contain minisatellite-like sequences, none was found to be hypermutable. Since all hypermutable minisatellites contain internal variants, they provide extremely informative systems for analyzing the complex turnover processes that occur at this class of tandem repeat. Minisatellite variant repeat mapping by PCR (MVR-PCR) has been extensively used to chart the interspersion patterns of variant repeats along the array, which provides details on the structure of the alleles before and after mutation. Studies have revealed distinct mutation processes operating in somatic and germline cells. Somatic instability detected in blood DNA shows simple and rare intra-allelic events two to three orders of magnitude lower than in sperm. In contrast, complex inter-allelic conversion-like events occur in the germline.[6] Additional analyses of DNA sequences flanking human minisatellites have also revealed an intense and highly localized meiotic crossover hotspot that is centered upstream of the unstable side of minisatellite arrays. Repeat turnover therefore appears to be controlled by recombinational activity in DNA that flanks the repeat array and results in a polarity of mutation. These findings have suggested that minisatellites most probably evolved as bystanders of localized meiotic recombination hotspots in the human genome.
Application Since the fortuitous discovery of the first human minisatellite in 1980 by A.R. Wyman and R. White[30] and especially the discovery that the extreme polymorphism of minisatellites made them superb for DNA fingerprinting by Alec Jeffreys,[31] this class of repeats has been an intense focus of studies that have addressed the turnover mechanisms that provoke their instability. Due to their high level of polymorphism, minisatellites have been extensively used for DNA fingerprinting as well as for genetic markers in linkage analysis and population studies.
Minisatellites have also been implicated as regulators of gene expression (e.g., at levels of transcription, alternative splicing, or imprint control) or as part of bona fide open reading frames.
Microsatellites
Microsatellites, also known as Simple Sequence Repeats (SSRs), or sometimes Short Tandem Repeats (STRs), are repeating sequences of 1-6 base pairs of DNA. Microsatellites are typically neutral and co-dominant. They are used as molecular markers in genetics, for kinship, population and other studies. They can also be used to study gene duplication or deletion.
One common example of a microsatellite is a (CA)n repeat, where n is variable between alleles. These markers often present high levels of inter- and intra-specific polymorphism, particularly when tandem repeats number ten or greater. The repeated sequence is often simple, consisting of two, three or four nucleotides (di-, tri-, and tetranucleotide repeats respectively), and can be repeated 10 to 100 times. CA nucleotide repeats are very frequent in human and other genomes, and are present every few thousand base pairs. As there are often many alleles present at a microsatellite locus, genotypes within pedigrees are often fully informative, in that the progenitor of a particular allele can often be identified. In this way, microsatellites are ideal for determining paternity, population genetic studies and recombination mapping. It is also the only molecular marker to provide clues about which alleles are more closely related. Microsatellites owe their variability to an increased rate of mutation compared to other neutral regions of DNA. These high rates of mutation can be explained most frequently by slipped strand mispairing (slippage) during DNA replication on a single DNA strand. Mutation may also occur during recombination during meiosis.[4] Some errors in slippage are rectified by proofreading mechanisms within the nucleus, but some mutations can escape repair. The size of the repeat unit, the number of repeats and the presence of variant repeats are all factors, as well as the frequency of transcription in the area of the DNA repeat. Interruption of microsatellites, perhaps due to mutation, can result in reduced polymorphism. However, this same mechanism can occasionally lead to incorrect amplification of microsatellites; if slippage occurs early on during PCR, microsatellites of incorrect lengths can be amplified.
Limitations
Microsatellites have proved to be versatile molecular markers, particularly for population analysis, but they are not without limitations. Microsatellites developed for particular species can often be applied to closely related species, but the percentage of loci that successfully amplify may decrease with increasing genetic distance. Point mutation in the primer annealing sites in such species may lead to the occurrence of ‘null alleles’, where microsatellites fail to amplify in PCR assays.[32] Null alleles can be attributed to several phenomena. Sequence divergence in flanking regions can lead to poor primer annealing, especially at the 3’ section, where extension commences; preferential amplification of particular size alleles due to the competitive nature of PCR can lead to heterozygous individuals being scored for homozygosity (partial null). PCR failure may result when particular loci fail to amplify, whereas others amplify more efficiently and may appear homozygous on a gel assay, when they are in reality heterozygous in the genome. Null alleles complicate the interpretation of microsatellite allele frequencies and thus make estimates of relatedness faulty. Furthermore, stochastic effects of sampling that occurs during mating may change allele frequencies in a way that is very similar to the effect of null alleles; an excessive frequency of homozygotes causing deviations from Hardy-Weinberg equilibrium expectations. Since null alleles are a technical problem and sampling effects that occur during mating are a real biological property of a population, it is often very important to distinguish between them if excess homozygotes are observed.
When using microsatellites to compare species, homologous loci may be easily amplified in related species, but the number of loci that amplify successfully during PCR may decrease with increased genetic distance between the species in question. Mutation in microsatellite alleles is biased in the sense that larger alleles contain more bases, and are therefore likely to be mistranslated in DNA replication. Smaller alleles also tend to increase in size, whereas larger alleles tend to decrease in size, as they may be subject to an upper size limit; this constraint has been determined but possible values have not yet been specified. If there is a large size difference between individual alleles, then there may be increased instability during recombination at meiosis. In tumour cells, where controls on replication may be damaged, microsatellites may be gained or lost at an especially high frequency during each round of mitosis. Hence a tumour cell line might show a different genetic fingerprint from that of the host tissue.
Mechanisms for change
The most common cause of length changes in short sequence repeats is replication slippage, caused by mismatches between DNA strands while being replicated during meiosis. Typically, slippage in each microsatellite occurs about once per 1,000 generations (Weber 1993). Slippage changes in repetitive DNA are orders of magnitude more common than point mutations in other parts of the genome. Most slippage results in a change of just one repeat unit, and slippage rates vary for different repeat unit sizes, and within different species.
Short sequence repeats are distributed throughout the genome. Presumably, their most probable means of expression will vary, depending on their location.
In proteins
In mammals, 20% to 40% of proteins contain repeating sequences of amino acids caused by short sequence repeats. Most of the short sequence repeats within protein-coding portions of the genome have a repeating unit of three nucleotides, since that length will not cause frame-shift mutations (Sutherland 1995). Each trinucleotide repeating sequence is transcribed into a repeating series of the same amino acid. In yeasts, the most common repeated amino acids are glutamine, glutamic acid, asparagine, aspartic acid and serine. These repeating segments can affect the physical and chemical properties of proteins, with the potential for producing gradual and predictable changes in protein action.
For example, length changes in tandemly repeating regions in the Runx2 gene lead to differences in facial length in domesticated dogs (Canis familiaris), with an association between longer sequence lengths and longer faces. This association also applies to a wider range of Carnivora species . Length changes in polyalanine tracts within the HoxA13 gene are linked to hand-foot-genital syndrome, a developmental disorder in humans . Length changes in other triplet repeats are linked to more than 40 neurological diseases in humans [33].
Evolutionary changes from replication slippage also occur in simpler organisms. For example, microsatellite length changes are common within surface membrane proteins in yeast, providing rapid evolution in cell properties. Specifically, length changes in the FLO1 gene control the level of adhesion to substrates. Short sequence repeats also provide rapid evolutionary change to surface proteins in pathenogenic bacteria, perhaps so they can keep up with immunological changes in their hosts. This is known as the Red Queen hypothesis. Length changes in short sequence repeats in a fungus (Neurospora crassa) control the duration of its circadian clock cycles [34].

Gene regulation
Length changes of microsatellites within promoters and other cis-regulatory regions can also change gene expression quickly, between generations. The human genome contains many (>16,000) short sequence repeats in regulatory regions, which provide ‘tuning knobs’ on the expression of many genes (Rockman 2002). Length changes in bacterial SSRs can affect fimbriae formation in Haemophilus influenza, by altering promoter spacing (Moxon 1994). Minisatellites are also linked to abundant variations in cis-regulatory control regions in the human genome . And microsatellites in control regions of the Vasopressin 1a receptor gene in voles influence their social behavior, and level of monogamy.
Within introns
Microsatellites within introns also influence phenotype, through means that are not currently understood. For example, a GAA triplet expansion in the first intron of the X25 gene appears to interfere with transcription, and causes Friedreich Ataxia (Bidichandani 1998). Tandem repeats in the first intron of the Asparagine synthetase gene are linked to acute lymphoblastic leukemia. A repeat polymorphism in the fourth intron of the NOS3 gene is linked to hypertension in a Tunisian population (Jemaa 2008). Reduced repeat lengths in the EGFR gene are linked with osteosarcomas.
Within transposons
Microsatellites are distributed throughout the genome . Almost 50% of the human genome is contained in various types of transposable elements (also called transposons, or ‘jumping genes’), and many of them contain repetitive DNA . It is probable that short sequence repeats in those locations are also involved in the regulation of gene expression [36].
Number of chromosomes in various organisms
[edit | edit source]Eukaryotes
[edit | edit source]These tables give the total number of chromosomes (including sex chromosomes) in a cell nucleus. For example, human cells are diploid and have 22 different types of autosome, each present as two copies, and two sex chromosomes. This gives 46 chromosomes in total. Other organisms have more than two copies of their chromosomes, such as bread wheat, which is hexaploid and has six copies of seven different chromosomes – 42 chromosomes in total.
|
|
|
Normal members of a particular eukaryotic species all have the same number of nuclear chromosomes (see the table). Other eukaryotic chromosomes, i.e., mitochondrial and plasmid-like small chromosomes, are much more variable in number, and there may be thousands of copies per cell.
Asexually reproducing species have one set of chromosomes, which are the same in all body cells. However, asexual species can be either haploid or diploid.
sexual reproduction|Sexually reproducing species have somatic cells (body cells), which are diploid [2n] having two sets of chromosomes, one from the mother and one from the father. Gametes, reproductive cells, are haploid [n]: They have one set of chromosomes. Gametes are produced by meiosis of a diploid germ line cell. During meiosis, the matching chromosomes of father and mother can exchange small parts of themselves (crossover), and thus create new chromosomes that are not inherited solely from either parent. When a male and a female gamete merge (fertilization), a new diploid organism is formed.
Some animal and plant species are polyploid [Xn]: They have more than two sets of homologous chromosomes. Plants important in agriculture such as tobacco or wheat are often polyploid, compared to their ancestral species. Wheat has a haploid number of seven chromosomes, still seen in some cultivars as well as the wild progenitors. The more-common pasta and bread wheats are polyploid, having 28 (tetraploid) and 42 (hexaploid) chromosomes, compared to the 14 (diploid) chromosomes in the wild wheat.[61]
Prokaryotes
[edit | edit source]Prokaryote species generally have one copy of each major chromosome, but most cells can easily survive with multiple copies.[62] For example, Buchnera, a symbiont of aphids has multiple copies of its chromosome, ranging from 10–400 copies per cell.[63] However, in some large bacteria, such as Epulopiscium fishelsoni up to 100,000 copies of the chromosome can be present.[64] Plasmids and plasmid-like small chromosomes are, as in eukaryotes, very variable in copy number. The number of plasmids in the cell is almost entirely determined by the rate of division of the plasmid – fast division causes high copy number, and vice versa.
chromosome anomaly, abnormality or aberration
[edit | edit source]When the chromosome's structure is altered. This can take several forms:
Deletions: A portion of the chromosome is missing or deleted. Known disorders in humans include Wolf-Hirschhorn syndrome, which is caused by partial deletion of the short arm of chromosome 4; and Jacobsen syndrome, also called the terminal 11q deletion disorder.
Duplications: A portion of the chromosome is duplicated, resulting in extra genetic material. Known human disorders include Charcot-Marie-Tooth disease type 1A which may be caused by duplication of the gene encoding peripheral myelin protein 22 (PMP22) on chromosome 17.
Translocations: When a portion of one chromosome is transferred to another chromosome. There are two main types of translocations. In a reciprocal translocation, segments from two different chromosomes have been exchanged. In a Robertsonian translocation, an entire chromosome has attached to another at the Centromere - in humans these only occur with chromosomes 13, 14, 15, 21 and 22.
Inversions: A portion of the chromosome has broken off, turned upside down and reattached, therefore the genetic material is inverted.
Rings: A portion of a chromosome has broken off and formed a circle or ring. This can happen with or without loss of genetic material.
Isochromosome: Formed by the mirror image copy of a chromosome segment including the centromere.
Chromosome instability syndromes are a group of disorders characterized by chromosomal instability and breakage. They often lead to an increased tendency to develop certain types of malignancies.
Chromosomal aberrations are disruptions in the normal chromosomal content of a cell and are a major cause of genetic conditions in humans, such as Down syndrome. Some chromosome abnormalities do not cause disease in carriers, such as translocations, or chromosomal inversions, although they may lead to a higher chance of birthing a child with a chromosome disorder. Abnormal numbers of chromosomes or chromosome sets, aneuploidy, may be lethal or give rise to genetic disorders. Genetic counseling is offered for families that may carry a chromosome rearrangement.
The gain or loss of DNA from chromosomes can lead to a variety of genetic disorders. Human examples include:
Cri du chat, which is caused by the deletion of part of the short arm of chromosome 5. "Cri du chat" means "cry of the cat" in French, and the condition was so-named because affected babies make high-pitched cries that sound like those of a cat. Affected individuals have wide-set eyes, a small head and jaw, moderate to severe mental health issues, and are very short.
Down syndrome, usually is caused by an extra copy of chromosome 21 (trisomy 21). Characteristics include decreased muscle tone, stockier build, asymmetrical skull, slanting eyes and mild to moderate developmental disability.[49]
Edwards syndrome, which is the second-most-common trisomy; Down syndrome is the most common. It is a trisomy of chromosome 18. Symptoms include motor retardation, developmental disability and numerous congenital anomalies causing serious health problems. Ninety percent die in infancy; however, those that live past their first birthday usually are quite healthy thereafter. They have a characteristic clenched hands and overlapping fingers.
Idic15, abbreviation for Isodicentric 15 on chromosome 15; also called the following names due to various researches, but they all mean the same; IDIC(15), Inverted duplication 15, extra Marker, Inv dup 15, partial tetrasomy 15
Jacobsen syndrome, also called the terminal 11q deletion disorder. This is a very rare disorder. Those affected have normal intelligence or mild developmental disability, with poor expressive language skills. Most have a bleeding disorder called Paris-Trousseau syndrome.
Klinefelter's syndrome (XXY). Men with Klinefelter syndrome are usually sterile, and tend to have longer arms and legs and to be taller than their peers. Boys with the syndrome are often shy and quiet, and have a higher incidence of speech delay and dyslexia. During puberty, without testosterone treatment, some of them may develop gynecomastia.
Patau Syndrome, also called D-Syndrome or trisomy-13. Symptoms are somewhat similar to those of trisomy-18, but they do not have the characteristic hand shape.
Small supernumerary marker chromosome. This means there is an extra, abnormal chromosome. Features depend on the origin of the extra genetic material. Cat-eye syndrome and isodicentric chromosome 15 syndrome (or Idic15) are both caused by a supernumerary marker chromosome, as is Pallister-Killian syndrome.
Triple-X syndrome (XXX). XXX girls tend to be tall and thin. They have a higher incidence of dyslexia.
Turner syndrome (X instead of XX or XY). In Turner syndrome, female sexual characteristics are present but underdeveloped. People with Turner syndrome often have a short stature, low hairline, abnormal eye features and bone development and a "caved-in" appearance to the chest.
XYY syndrome. XYY boys are usually taller than their siblings. Like XXY boys and XXX girls, they are somewhat more likely to have learning difficulties. Wolf-Hirschhorn syndrome, which is caused by partial deletion of the short arm of chromosome 4. It is characterized by severe growth retardation and severe to profound mental health issues.
Lampbrush chromosomes
[edit | edit source]
Lampbrush chromosomes (first seen by Flemming in 1882) are a special form of chromosomes that are found in the growing oocytes (immature eggs) of most animals, except mammals. Lampbrush chromosomes of tailed and tailless amphibians, birds and insects are described best of all . Chromosomes transform into the lampbrush form during the diplotene stage of meiotic prophase I due to an active transcription of many genes. They are highly extended meiotic halve-bivalents, each consisting of 2 sister chromatids. Lampbrush chromosomes are clearly visible even in the light microscope, where they are seen to be organized into a series of chromomeres with large chromatin loops extended laterally. Amphibian and avian lampbrush chromosomes can be microsurgically isolated form oocyte nucleus (germinal vesicle) with either forceeps or needles.A given loop always contains the same DNA sequence, and it remains extended in the same manner as the oocytes grows. These chromosomes are producing large amounts of RNA for the oocyte, and most of the genes present in the DNA loops are being actively expressed. Each lateral loop contains one or several transcription units with polarized RNP-matrix coating the DNA axis of the loop. The majority of the DNA, however, is not in loops but remains highly condensed in the chromomeres on the axis, where genes are generally not expressed. It is thought that the interphase chromosomes of all eukaryotes are similarly arranged in loops. Although these loops are normally too small and fragile to be easily observed in a light microscope, other methods can be used to infer their presence. For example, it has become possible to assess the frequency with which two loci along an interphase chromosome are paired with each other, thus revealing candidates for the sites on chromatin that form the closely apposed bases of loop structures. These experiments and others suggest that the DNA in human chromosomes is organized into loops of different lengths. A typical loop might contain between 50,000 and 200,000 nucleotide pairs of DNA, although loops of a million nucleotide pairs have also been suggested.[65]
Giant chromosomes in the lampbrush form are useful model for studying chromosome organization, genome function and gene expression during meiotic prophase, since they allow the individual transcription units to be visualized. Moreover lampbrush chromosomes are widely used for high-resolution mapping of DNA sequences and construction of detail cytological maps of individual chromosomes.[66] To increase cell volume, some specialized cells undergo repeated rounds of DNA replication without cell division (endomitosis), forming a giant polytene chromosome. Polytene chromosomes form when multiple rounds of replication produce many sister chromatids that remain synapsed together. In addition to increasing the volume of the cells' nuclei and causing cell expansion, polytene cells may also have a metabolic advantage as multiple copies of genes permits a high level of gene expression. In Drosophila melanogaster, for example, the chromosomes of the larval salivary glands undergo many rounds of endoreplication, to produce large amounts of glue before pupation.[67]
Polytene chromosomes
[edit | edit source]Polytene chromosomes have characteristic light and dark banding patterns that can be used to identify chromosomal rearrangements and deletions. Dark banding frequently corresponds to inactive chromatin, whereas light banding is usually found at areas with higher transcriptional activity. The banding patterns of the polytene chromosomes of Drosophila melanogaster were sketched in 1935 by Calvin B. Bridges, in such detail that his maps are still widely used today. The banding patterns of the chromosomes are especially helpful in research, as they provide an excellent visualization of transcriptionally active chromatin and general chromatin structure. Chromosome puffs are diffused uncoiled regions of the polytene chromosome that are sites of RNA transcription. A Balbiani ring is a large chromosome puff. Polytene chromosomes were originally observed in the larval salivary glands of Chironomus midges by Balbiani in 1881, but the hereditary nature of these structures was not confirmed until they were studied in Drosophila melanogaster in the early 1930s by Emil Heitz and Hans Bauer. They are known to occur in secretory tissues of other dipteran insects such as the Malpighian tubules of Sciara and also in protists, plants, mammals, or in cells from other insects. Some of the largest polytene chromosomes described thus far occur in larval salivary gland cells of the Chironomid genus Axarus. Another form of chromosomal enlargement that provides for increased transcription is the lampbrush chromosome. Polytene chromosomes are also used to identify the species of Chironomid larvae that are notoriously difficult to identify. Each morphologically distinct group of larvae consists of a number of morphologically identical (sibling) species that can only be identified by rearing adult males or by cytogenetic analysis of the polytene chromosomes of the larvae. Karyotypes are used to confirm the presence of specific species and to study genetic diversity in species with a wide range.[68]
B chromosomes
[edit | edit source]In addition to the normal karyotype, wild populations of many animal, plant, and fungi species contain B chromosomes (also known as supernumerary or accessory chromosomes). By definition, these chromosomes are not essential for the life of a species, and are lacking in some (usually most) of the individuals. Thus a population would consist of individuals with 0, 1, 2, 3 (etc) supernumeraries. Most B chromosomes are mainly or entirely heterochromatic, (and so would be largely non-coding) but some, such as the B chromosomes of maize, contain sizeable euchromatic segments. In general it seems unlikely that supernumeraries would persist in a species unless there was some positive adaptive advantage, which in a few cases has been identified. For instance, the British grasshopper Myrmeleotettix maculatus has two structural types of B chromosomes: metacentrics and submetacentrics. The supernumeraries, which have a satellite DNA, occur in warm, dry environments, and are scarce or absent in humid, cooler localities. In plants there is a tendency for B chromosomes to be present in the germ-line, but to be lost from other tissues such as root tips and leaves. There is evidence of deleterious effects of supernumeraries on pollen fertility, and favourable effects or associations with particular habitats are also known in a number of species. The evolutionary origin of supernumerary chromosomes is obscure, but presumably they must have been derived from heterochromatic segments of normal chromosomes in the remote past. In general "we may regard supernumeraries as a very special category of genetic polymorphism which, because of manifold types of accumulation mechanisms, does not obey the ordinary Mendelian laws of inheritance." B chromosomes may play a positive role on normal A chromosomes in some circumstances. The B chromosomes suppress homologous pairing which reduces multiple pairing between homologous chromosomes in allopolyploids. Bivalent pairing is ensured by a gene on chromosome 5 of the B genome Phlocus. The B chromosomes also have the following effects on A chromosomes: increases asymmetry chiasma distribution increases crossing over and recombination frequencies: increases variation cause increased unpaired chromosomes: infertility B chromosomes have tendency to accumulate in meiotic cell products resulting in an increase of B number over generations. However this effect is counterbalanced for selection against infertility. B chromosomes are not to be confused with marker chromosomes or additional copies of normal chromosomes as they occur in Trisomies.[69][70]
Supernumerary chromosomes in fungi
[edit | edit source]Chromosome polymorphisms are very common among fungi. Different isolates of the same species often have a different chromosome number, with some of these additional chromosomes being unnecessary for normal growth in culture. The extra chromosomes are known as conditionally dispensable, or supernumerary, because they are dispensable for certain situations, but may confer a selective advantage under different environments. Supernumerary chromosomes do not carry genes that are necessary for basic fungal growth, but may have some functional significance. For example, it has been discovered that the supernumerary chromosome of the pea pathogen Haematonectria haematococca carries genes that are important to the disease-causing capacity of the fungus. This supernumerary DNA was found to code for a group of enzymes that metabolize toxins, known as phytoalexins, that are secreted by the plant's immune system. It is possible that these supernumerary elements originated in horizontal gene transfer events because sequence analysis often indicates that they have a different evolutionary history from essential chromosomal DNA.[71]
Refrences
[edit | edit source]- ↑ http://en.wikipedia.org/w/index.php?title=Chromosome&oldid=424607651
- ↑ http://en.wikipedia.org/w/index.php?title=Chromosome&oldid=424607651
- ↑ http://en.wikipedia.org/w/index.php?title=Chromosome&oldid=424607651
- ↑ http://en.wikipedia.org/w/index.php?title=Chromatin&oldid=423073917
- ↑
Bernstein, B.E., T.S. Mikkelsen, X. Xie, M. Kamal, D.J. Huebert, J. Cuff, B. Fry, A. Meissner, M. Wernig, K. Plath, R. Jaenisch, A. Wagschal, R. Feil, S.L. Schreiber & E.S. Lander (2006). "A bivalent chromatin structure marks key developmental genes in embryonic stem cells". Cell. 125 (2): 315–26. doi:10.1016/j.cell.2006.02.041. ISSN 0092-8674. PMID 16630819.
{{cite journal}}
: Unknown parameter|month=
ignored (help)CS1 maint: multiple names: authors list (link) - ↑ Portoso M and Cavalli G (2008). "The Role of RNAi and Noncoding RNAs in Polycomb Mediated Control of Gene Expression and Genomic Programming". RNA and the Regulation of Gene Expression: A Hidden Layer of Complexity. Caister Academic Press. ISBN 978-1-904455-25-7.
{{cite book}}
: Unknown parameter|chapterurl=
ignored (|chapter-url=
suggested) (help) - ↑ http://en.wikipedia.org/w/index.php?title=Histone&oldid=423138985
- ↑ http://en.wikipedia.org/w/index.php?title=Histone&oldid=423138985
- ↑ Clarke HJ (1992). "Nuclear and chromatin composition of mammalian gametes and early embryos". Biochem. Cell Biol. 70 (10-11): 856–66.
- ↑
Robinson DJ, Fairall L, Huynh VA, Rhodes D. (2006). "EM measurements define the dimensions of the "30-mm" chromatin fiber: evidence for a compact, interdigitated structure". PNAS. 103 (17): 6506–11. doi:10.1073/pnas.0601212103. PMC 1436021. PMID 16617109.
{{cite journal}}
: Unknown parameter|month=
ignored (help)CS1 maint: multiple names: authors list (link) - ↑
Wong H, Victor JM, Mozziconacci J. (2007). "An all-atom model of the chromatin fiber containing linker histones reveals a versatile structure tuned by the nucleosomal repeat length". PLoS ONE. 2 (9): e877. doi:10.1371/journal.pone.0000877. PMC 1963316. PMID 17849006.
{{cite journal}}
: Unknown parameter|month=
ignored (help)CS1 maint: multiple names: authors list (link) - ↑ Vega.sanger.ad.uk, all data in this table was derived from this database, November 11, 2008.
- ↑ Griffiths, Anthony J. F. (1999). An Introduction to genetic analysis. New York: W.H. Freeman. ISBN 071673771X.
- ↑ http://en.wikipedia.org/w/index.php?title=Nucleoid&oldid=422241758
- ↑ http://en.wikipedia.org/w/index.php?title=Nucleoid&oldid=422241758
- ↑ http://en.wikipedia.org/w/index.php?title=Circular_bacterial_chromosome&oldid=422751175
- ↑ Myka JL, Lear TL, Houck ML, Ryder OA, Bailey E (2003). "FISH analysis comparing genome organization in the domestic horse (Equus caballus) to that of the Mongolian wild horse (E. przewalskii)". Cytogenet. Genome Res. 102 (1–4): 222–5. doi:10.1159/000075753. PMID 14970707.
{{cite journal}}
: CS1 maint: multiple names: authors list (link) - ↑ 5.2 KARYOTYPES, CHROMOSOMES, AND TRANSLOCATIONS
Waterston RH, Lindblad-Toh K, Birney E; et al. (2002). "Initial sequencing and comparative analysis of the mouse genome". Nature. 420 (6915): 520–62. doi:10.1038/nature01262. PMID 12466850.{{cite journal}}
: Explicit use of et al. in:|author=
(help); Unknown parameter|month=
ignored (help)CS1 maint: multiple names: authors list (link) - ↑ Dernburg AF (2001). "Here, there, and everywhere: kinetochore function on holocentric chromosomes". J. Cell Biol. 153 (6): F33–8. doi:10.1083/jcb.153.6.F33. PMC 2192025. PMID 11402076.
{{cite journal}}
: Unknown parameter|month=
ignored (help) - ↑ Londono-Vallejo, Arturo, and Raymund Wellinger. "Elsevier: Article Locator." ScienceDirect.com | Search through over 11 million science, health, medical journal full text articles and books.. N.p., n.d. Web. 6 Dec. 2012. <http://www.sciencedirect.com/science/article/pii/S0968000412000722#>.
- ↑ Lundblad, 2000; Ferreira et al., 2004
- ↑ Maloy, Stanley (July 12, 2002). "Bacterial Chromosome Structure". Retrieved 2008-06-22.
- ↑ Shampay.J., Szostak,J.W. and Blackburn,E.H. (1984) Nature, 310, 154-157
- ↑ Blackburn, 2001; Smogorzewska and de Lange, 2004; Cech, 2004; De Lange et al., 2005; Kota and Runge, 1999
- ↑ Griffith J, Comeau L, Rosenfield S, Stansel R, Bianchi A, Moss H, de Lange T (1999). "Mammalian telomeres end in a large duplex loop". Cell 97 (4): 503–14.
- ↑ Burge S, Parkinson G, Hazel P, Todd A, Neidle S (2006). "Quadruplex DNA: sequence, topology and structure". Nucleic Acids Res 34 (19): 5402–15.
- ↑ a b c Eisenberg DTA (2011). "An evolutionary review of human telomere biology: The thrifty telomere hypothesis and notes on potential adaptive paternal effects". American Journal of Human Biology. doi:10.1002/ajhb.21127.
- ↑ Eisenberg DTA (2011). "An evolutionary review of human telomere biology: The thrifty telomere hypothesis and notes on potential adaptive paternal effects". American Journal of Human Biology. doi:10.1002/ajhb.21127.
- ↑ Buard J, Vergnaud G Complex recombination events at the hypermutable minisatellite CEB1 (D2S90)EMBO J.(1994 )vol.13 p3203–10
- ↑ Wyman AR, White R (1980). "A highly polymorphic locus in human DNA". Proc. Natl. Acad. Sci. U.S.A. 77 (11): 6754–8. doi:10.1073/pnas.77.11.6754. PMC 350367. PMID 6935681.
{{cite journal}}
: Unknown parameter|month=
ignored (help) - ↑ Jeffreys AJ (2005). "Genetic fingerprinting". Nat. Med. 11 (10): 1035–9. doi:10.1038/nm1005-1035. PMID 16211029.
{{cite journal}}
: Unknown parameter|month=
ignored (help) - ↑ Dakin, EE; Avise, JC (2004). "Microsatellite null alleles in parentage analysis". Heredity. 93 (5): 504–509. doi:10.1038/sj.hdy.6800545. PMID 15292911.
{{cite journal}}
: More than one of|author=
and|last1=
specified (help) - ↑ Pearson, C. E., et al. 2005. Repeat instability: mechanisms of dynamic mutations. Nat. Rev. Gen. 6:729-742.
- ↑ Michael, T. P., et al. 2008. Simple sequence repeats provide a substrate for phenotypic variation in the Neurospora crassa circadian clock. PLoS One 2:e795.
- ↑ Bolzer et al., (2005) Three-Dimensional Maps of All Chromosomes in Human Male Fibroblast Nuclei and Prometaphase Rosettes. PLoS Biol 3(5): e157 DOI: 10.1371/journal.pbio.0030157
- ↑ Tomilin, N. V. 2008. Regulation of mammalian gene expression by retroelements and non-coding tandem repeats. BioEssays 30:338-348
- ↑ Armstrong SJ, Jones GH (2003). "Meiotic cytology and chromosome behaviour in wild-type Arabidopsis thaliana". J. Exp. Bot. 54 (380): 1–10. doi:10.1093/jxb/54.380.1. PMID 12456750.
{{cite journal}}
: Unknown parameter|month=
ignored (help) - ↑ Gill BS, Kimber G (1974). "The Giemsa C-banded karyotype of rye". Proc. Natl. Acad. Sci. U.S.A. 71 (4): 1247–9. doi:10.1073/pnas.71.4.1247. PMC 388202. PMID 4133848.
{{cite journal}}
: Unknown parameter|month=
ignored (help) - ↑ Kato A, Lamb JC, Birchler JA (2004). "Chromosome painting using repetitive DNA sequences as probes for somatic chromosome identification in maize". Proc. Natl. Acad. Sci. U.S.A. 101 (37): 13554–9. doi:10.1073/pnas.0403659101. PMC 518793. PMID 15342909.
{{cite journal}}
: Unknown parameter|month=
ignored (help)CS1 maint: multiple names: authors list (link) - ↑ a b c Dubcovsky J, Luo MC, Zhong GY; et al. (1996). "Genetic map of diploid wheat, Triticum monococcum L., and its comparison with maps of Hordeum vulgare L". Genetics. 143 (2): 983–99. PMC 1207354. PMID 8725244.
{{cite journal}}
: Explicit use of et al. in:|author=
(help)CS1 maint: multiple names: authors list (link) - ↑ Kenton A, Parokonny AS, Gleba YY, Bennett MD (1993). "Characterization of the Nicotiana tabacum L. genome by molecular cytogenetics". Mol. Gen. Genet. 240 (2): 159–69. doi:10.1007/BF00277053. PMID 8355650.
{{cite journal}}
: Unknown parameter|month=
ignored (help)CS1 maint: multiple names: authors list (link) - ↑ Leitch IJ, Soltis DE, Soltis PS, Bennett MD (2005). "Evolution of DNA amounts across land plants (embryophyta)". Ann. Bot. 95 (1): 207–17. doi:10.1093/aob/mci014. PMID 15596468.
{{cite journal}}
: CS1 maint: multiple names: authors list (link) - ↑ Umeko Semba, Yasuko Umeda, Yoko Shibuya, Hiroaki Okabe, Sumio Tanase and Tetsuro Yamamoto (2004). "Primary structures of guinea pig high- and low-molecular-weight kininogens". International Immunopharmacology. 4 (10–11): 1391–1400. doi:10.1016/j.intimp.2004.06.003. PMID 15313436.
{{cite journal}}
: CS1 maint: multiple names: authors list (link) - ↑ "The Genetics of the Popular Aquarium Pet - Guppy Fish". Retrieved 2009-12-06.
- ↑ Vitturi R, Libertini A, Sineo L; et al. (2005). "Cytogenetics of the land snails Cantareus aspersus and C. mazzullii (Mollusca: Gastropoda: Pulmonata)". Micron. 36 (4): 351–7. doi:10.1016/j.micron.2004.12.010. PMID 15857774.
{{cite journal}}
: Explicit use of et al. in:|author=
(help)CS1 maint: multiple names: authors list (link) - ↑ Vitturi R, Colomba MS, Pirrone AM, Mandrioli M (2002). "rDNA (18S-28S and 5S) colocalization and linkage between ribosomal genes and (TTAGGG)(n) telomeric sequence in the earthworm, Octodrilus complanatus (Annelida: Oligochaeta: Lumbricidae), revealed by single- and double-color FISH". J. Hered. 93 (4): 279–82. doi:10.1093/jhered/93.4.279. PMID 12407215.
{{cite journal}}
: CS1 maint: multiple names: authors list (link) - ↑ Nie W, Wang J, O'Brien PC; et al. (2002). "The genome phylogeny of domestic cat, red panda and five mustelid species revealed by comparative chromosome painting and G-banding". Chromosome Res. 10 (3): 209–22. doi:10.1023/A:1015292005631. PMID 12067210.
{{cite journal}}
: Explicit use of et al. in:|author=
(help)CS1 maint: multiple names: authors list (link) - ↑ a b Romanenko, Svetlana A (2006-12). "Reciprocal chromosome painting between three laboratory rodent species". Mammalian Genome: Official Journal of the International Mammalian Genome Society. 17 (12): 1183–1192. doi:10.1007/s00335-006-0081-z. ISSN 0938-8990. PMID 17143584. Retrieved 2009-10-14.
{{cite journal}}
: Check date values in:|date=
(help); Unknown parameter|coauthors=
ignored (|author=
suggested) (help) - ↑ a b A Comparison of the chromosomes of the rat and mouse with reference to the question of chromosome homology in mammals
- ↑ Hayes, H. (2002). "Establishment of an R-banded rabbit karyotype nomenclature by FISH localization of 23 chromosome-specific genes on both G- and R-banded chromosomes". Cytogenetic and Genome Research. 98 (2–3): 199–205. doi:10.1159/000069807. ISSN 1424-859X. PMID 12698004. Retrieved 2009-10-14.
{{cite journal}}
: Unknown parameter|coauthors=
ignored (|author=
suggested) (help) - ↑ T.J. Robinson, F. Yang, W.R. Harrison (2002). "Chromosome painting refines the history of genome evolution in hares and rabbits (order Lagomorpha)". Cytogenic and Genetic Research. 96 (1–4): 223–227. doi:10.1159/000063034. PMID 12438803.
{{cite journal}}
: CS1 maint: multiple names: authors list (link) - ↑ "Rabbits, Hares and Pikas. Status Survey and Conservation Action Plan". pp. 61–94.
{{cite web}}
:|section=
ignored (help) - ↑ a b De Grouchy J (1987). "Chromosome phylogenies of man, great apes, and Old World monkeys". Genetica. 73 (1–2): 37–52. PMID 3333352.
- ↑ Houck ML, Kumamoto AT, Gallagher DS, Benirschke K (2001). "Comparative cytogenetics of the African elephant (Loxodonta africana) and Asiatic elephant (Elephas maximus)". Cytogenet. Cell Genet. 93 (3–4): 249–52. doi:10.1159/000056992. PMID 11528120.
{{cite journal}}
: CS1 maint: multiple names: authors list (link) - ↑ Wayne RK, Ostrander EA (1999). "Origin, genetic diversity, and genome structure of the domestic dog". Bioessays. 21 (3): 247–57. doi:10.1002/(SICI)1521-1878(199903)21:3<247::AID-BIES9>3.0.CO;2-Z. PMID 10333734.
- ↑ Burt DW (2002). "Origin and evolution of avian microchromosomes". Cytogenet. Genome Res. 96 (1–4): 97–112. doi:10.1159/000063018. PMID 12438785.
- ↑ Ciudad J, Cid E, Velasco A, Lara JM, Aijón J, Orfao A (2002). "Flow cytometry measurement of the DNA contents of G0/G1 diploid cells from three different teleost fish species". Cytometry. 48 (1): 20–5. doi:10.1002/cyto.10100. PMID 12116377.
{{cite journal}}
: CS1 maint: multiple names: authors list (link) - ↑ Yasukochi Y, Ashakumary LA, Baba K, Yoshido A, Sahara K (2006). "A second-generation integrated map of the silkworm reveals synteny and conserved gene order between lepidopteran insects". Genetics. 173 (3): 1319–28. doi:10.1534/genetics.106.055541. PMC 1526672. PMID 16547103.
{{cite journal}}
: CS1 maint: multiple names: authors list (link) - ↑ Itoh, Masahiro (1969). "A COMPARATIVE KARYOTYPE STUDY IN FOURTEEN SPECIES OF BIRDS". The Japanese journal of genetics. 44 (3): 163–170. doi:10.1266/jjg.44.163. ISSN 1880-5787. Retrieved 2009-10-14.
{{cite journal}}
: Unknown parameter|coauthors=
ignored (|author=
suggested) (help) - ↑ Smith J, Burt DW (1998). "Parameters of the chicken genome (Gallus gallus)". Anim. Genet. 29 (4): 290–4. doi:10.1046/j.1365-2052.1998.00334.x. PMID 9745667.
- ↑ Sakamura, T. (1918), Kurze Mitteilung uber die Chromosomenzahlen und die Verwandtschaftsverhaltnisse der Triticum-Arten. Bot. Mag., 32: 151-154.
- ↑ Charlebois R.L. (ed) 1999. Organization of the prokaryote genome. ASM Press, Washington DC.
- ↑ Komaki K, Ishikawa H (2000). "Genomic copy number of intracellular bacterial symbionts of aphids varies in response to developmental stage and morph of their host". Insect Biochem. Mol. Biol. 30 (3): 253–8. doi:10.1016/S0965-1748(99)00125-3. PMID 10732993.
{{cite journal}}
: Unknown parameter|month=
ignored (help) - ↑ Mendell JE, Clements KD, Choat JH, Angert ER (2008). "Extreme polyploidy in a large bacterium". Proc. Natl. Acad. Sci. U.S.A. 105 (18): 6730–4. doi:10.1073/pnas.0707522105. PMC 2373351. PMID 18445653.
{{cite journal}}
: Unknown parameter|month=
ignored (help)CS1 maint: multiple names: authors list (link) - ↑ http://en.wikipedia.org/w/index.php?title=Lampbrush_chromosome&oldid=423150981
- ↑ http://en.wikipedia.org/w/index.php?title=Lampbrush_chromosome&oldid=423150981
- ↑ http://en.wikipedia.org/w/index.php?title=Lampbrush_chromosome&oldid=423150981
- ↑ http://en.wikipedia.org/w/index.php?title=Polytene_chromosome&oldid=422375538
- ↑ http://en.wikipedia.org/w/index.php?title=B_chromosome&oldid=424347426
- ↑ Camacho, J.P.M. (2004). "B Chromosomes in the Eukaryote Genome". Cytogenetics and Genome Research 106 (2-4). (Special issue)
- ↑ http://en.wikipedia.org/w/index.php?title=B_chromosome&oldid=424347426